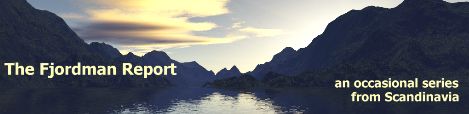
The noted blogger Fjordman is filing this report via Gates of Vienna.
For a complete Fjordman blogography, see The Fjordman Files. There is also a multi-index listing here.
This essay is the fifth of six parts. Part 4 is here.

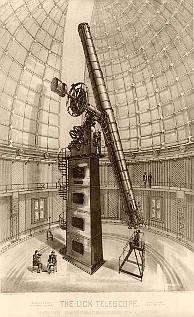
Even with the fine lenses made by the brothers Huygens, by the 1660s refracting telescopes had reached the limits of what was reasonable in the absence of achromatic lenses. Newton’s discovery that sunlight is made up of rays of different refrangibilities was demonstrated in his Opticks from 1704. Sunlight passed through a glass prism yielded a spectrum of rays of different colors which, if reunited by a second prism, again produced white light. The different refrangibilities of rays of different colors make lenses cast colored images. Newton thought, mistakenly, that this evil could not be cured, and made the first practical reflecting telescope in 1668. It was widely believed that Newton had proved the impossibility of removing chromatic aberration and this delayed the development of achromatic lenses.
The English lawyer and amateur optician Chester Moore Hall (1703—1771) proved through a series of experiments that different sorts of glass (crown and flint) could be combined to produce an achromatic combination. He had at least two achromatic telescopes made after 1730 in collaboration with the optician George Bass, and told the well-known English instrument maker John Dollond (1706-1761) of his success in the 1750s. His son Peter Dollond (1730-1820) had recently started making optical instruments, and John’s reputation grew rapidly. The brilliant Swiss mathematician Leonhard Euler saw the potential in achromatism and wrote to Dollond about it.
Dollond at first doubted the possibility of making achromatic lenses, but the Swedish scientist Samuel Klingenstierna (1698-1765) found errors in Newton’s theories of refraction, published his theoretical account of this in 1754 and sent his geometrical notes to Dollond. John Dollond in 1757 managed to produce achromatic doublets by a combination of different forms of glass and became the first to patent the method. This quickly led to much-improved refracting telescopes, but in the long run reflecting telescopes would still win out.
Great improvements took place in optics during the nineteenth century, especially in Germany at the firm of Carl Zeiss (1816—1888), supported by the theoretician Ernst Abbe (1840—1905) and in collaboration with the scientific glassworks of Otto Schott (1851-1935). Justus von Liebig (1803—1873) and other chemists made contributions as well. John North again:
“The future of telescopes was with reflectors, and this simply because the glass at the center of lenses of the order of a meter in diameter is so thick that the absorption of light there is intolerable. Physical deformation under the glass’s weight is also a problem. A new medium was needed, however, to replace the unworkable speculum metal. Glass mirrors were not new, and glass grinding and polishing was a highly developed art, but early methods of silvering the glass were crude. In 1853, the German chemist Justus von Liebig devised a technique for depositing a thin and uniform layer of silver on a clean glass surface, from an aqueous solution of silver nitrate. The technique had been shown at the Great Exhibition of 1851 in London but was seemingly reinvented by Liebig, who brought it to the attention of a wide scientific circle. A few years afterwards, the renowned Munich instrument maker Carl August von Steinheil of Munich, and Jean Bernard Léon Foucault of Paris, both made use of the technique, depositing layers of silver on glass mirrors for use in telescope — at first in fairly small ones.”
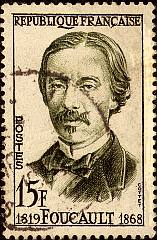
Newton supported the French atomist Pierre Gassendi’s corpuscular theory of light. The revived atomism of Gassendi had many supporters during the seventeenth century. The Englishman Thomas Hobbes, remembered mainly for his work Leviathan from 1651 written during the English Civil War (1642—51) where he developed the concept of “the war of all against all” and the idea of the social contract, which would later be elaborated in very different ways by John Locke and Jean-Jacques Rousseau, used atomism as an inspiration for his political philosophy. Christiaan Huygens had practical experience from working with lenses and mirrors and like Descartes, but unlike Newton, supported a wave theory of light.
- - - - - - - - -
According to Gribbin, “Italian physicist Francesco Grimaldi (1618-1663), professor of mathematics at the Jesuit college in Bologna…studied light by letting a beam of sunlight into a darkened room through a small hole. He found that when the beam of light was passed through a second small hole and on to a screen, the image on the screen formed by the spot of light had coloured fringes and was slightly larger than it should be if the light had travelled in straight lines through the hole. He concluded (correctly) that the light had been bent outwards slightly as it passed through the hole, a phenomenon to which he gave the name ‘diffraction’. He also found that when a small object (such as a knife edge) was placed in the beam of light, the shadow cast by the object had coloured edges where light had been diffracted around the edge of the object and leaked into the shadow. This is direct evidence that light travels as a wave, and the same sort of effect can be seen when waves on the sea, or on a lake, move past obstructions or through gaps in obstructions.”
Because the wavelengths are so small, the effects are tiny and difficult to measure. Moreover, Grimaldi’s work was published two years after he died. There were a few scholars who supported a wave theory of light in the eighteenth century, most notably Leonhard Euler, yet Newton’s conception of light as a stream of particles dominated the field for a long time due to his great personal authority, as the man who had discovered the laws of universal gravity.
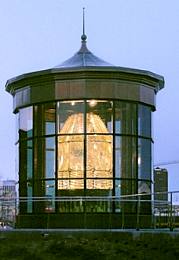
Young contributed to the study of vision as well, and theorized that the most sensitive points of the retina, which are connected directly to the brain, can detect three primary colors. The brain later mixes the sensations to create all possible colors. Individuals suffering from color blindness, a disorder first recognized during Young’s lifetime (the English scholar John Dalton published a scientific paper on the subject in 1798) have an abnormally low number of retinal cones which detect color.
This idea was developed further by the German physician and physicist Hermann von Helmholtz (1821-1894) and is called the Young-Helmholtz theory. Later experiments demonstrated that you can indeed make other colors by mixing the three primary colors red, green and blue. The existence of three types of cones, sensitive to different wavelengths of light, was confirmed in the late twentieth century. This trichromatic theory of color vision was later challenged by the German physiologist Ewald Hering (1834-1918), who proposed an alternative theory. We know today that the theories describe different parts of the process.
Traditionally, the human eye has been viewed as having two types of photoreceptor cells: rods and cones. Rods are more sensitive to light than cones and thus responsible for night vision, but do not distinguish color equally well. In the 1990s there were claims that a third type of retinal photoreceptors had been discovered, so-called photosensitive ganglion cells, but while they may affect some biological processes their role is apparently non-image-forming.
Helmholtz invented the ophthalmoscope which could examine the inside of the human eye. In the 1850s he studied color vision, inspired by Young’s and Maxwell’s work, and published Handbuch der Physiologischen Optik (Handbook of Physiological Optics) in 1860. He also made contributions to our understanding of the mechanisms of hearing and was familiar with the Theory of Colours (Zur Farbenlehre) from 1810 by Johann Wolfgang von Goethe (1749-1832). Goethe had a powerful mind and was one of the greatest writers in European history, but he was not a physicist and concerned himself mainly with color perception, hence his work influenced artists such as the Russian painter Wassily Kandinsky (1866-1944) and the English romantic painter J. M. W. Turner (1775-1851) more than scientists.
The trichromatic theory of color vision by Young and Helmholtz was later challenged by the German physiologist Ewald Hering (1834-1918), who proposed an alternative theory. We know today that the theories describe different parts of the process. Research during the late twentieth century confirmed that the human eye contains three types of color sensors, the photoreceptors, composed of so-called red, blue and green cones because of their absorption of light at those wavelengths.
The industrial research laboratories, another European innovation of the nineteenth century, were first applied to chemistry, which was used to analyze the properties of a wide range of known materials and understand how they could be improved by testing, measuring, analyzing and quantifying processes and products that already existed in metallurgy, textiles, etc. Eventually this led to the creation of entirely new products, the synthetic dye industry, synthetic textiles and to the era of plastics and synthetic fibers.
The idea of contact lenses had been suggested by Leonardo da Vinci, Descartes and others, but the first usable contact lenses were made in the nineteenth century. In 1887 a German glassblower, F.E. Muller, produced the first eye covering to be seen through and tolerated. In the following year, the physiologist Adolf Eugen Fick and the Paris optician Edouard Kalt simultaneously reported using contact lenses to correct optical defects. Although some contact lenses have been and still are being made of glass, the real breakthrough for the use of such lenses came in the twentieth century with the development of alternative materials. In 1936 the American optometrist William Feinbloom (1904-1985) introduced the use of plastic. The most important breakthrough came with the Czech chemists Otto Wichterle (1913—1998) and Drahoslav Lím (1925-2003) and their experiments with lenses made of a soft, water-absorbing plastic they developed. They published their work in the influential journal Nature in 1959. Soft contact lenses, which are thinner, lighter and more comfortable to wear than hard ones, were made commercially available from the 1970s onwards, and only then became a widely used alternative to spectacles.
A chemistry-based innovation of far greater importance to science than the invention of contact lenses was spectroscopy. The English chemist William Hyde Wollaston (1766-1828) noted in 1802 some dark features in the solar spectrum, but he didn’t follow this insight up. In 1814, the German physicist Joseph von Fraunhofer (1787—1826) independently discovered these dark features (absorption lines) in the optical spectrum of the Sun, which are now known as Fraunhofer lines. He carefully studied them and noted that they exist in the spectra of Venus and the stars as well, which meant that they had to be a property of the light itself. As so many times before, this optical advance was aided by advances in glassmaking.
In the 1780s a Swiss artisan, Pierre-Louis Guinand (1748-1824), began experimenting with the manufacture of flint glass, and in 1805 managed to produce a nearly flawless material. He passed on this secret to Fraunhofer, a skilled artisan working in the secularized Benedictine monastery of Benediktbeuern, who improved upon Guinand’s complex glass-stirring technique and managed to manufacture flint glass of unprecedented homogeneity in the 1810s and 1820s. Fraunhofer then began a more systematic study of the mysterious spectral lines. To the stronger ones he assigned the letters A to Z, a system which is still used today.
However, it was left to two other German scholars to prove the full significance of these lines. The birth of spectroscopy, the systematic study of the interaction of light with matter, came with the work of Robert Bunsen (1811—1899) and Gustav Kirchhoff (1824—1887). Cathy Cobb and Harold Goldwhite explain:
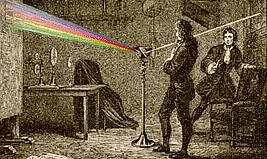
“Newton separated white light into component colors with a glass prism, then recombined it into white light by passing it through a second prism; the ancient texts of India report the use of flame color in chemical analysis (though the Indian savants were looking for poisons, not new elements); later chemists used flame colors as their only way of distinguishing sodium and potassium salts. The discovery to be exploited in the 1800s however was that elements in flames have spectra that show characteristic line patterns, and these line patterns can be measured and cataloged. German chemist Robert Bunsen….needed a steady, essentially colorless flame to analyze flame colors of salts in mineral waters, so he invented the laboratory burner named in his honor, the Bunsen burner….Gustav Robert Kirchhoff, a physicist and colleague at Heidelberg, suggested passing the light through a prism to spread out its component parts into a spectrum. Together the two workers assembled the flame, prism, lenses, and viewing tubes on a stand and produced the first spectrometer, and in very short order they used their spectrometer to identify the new elements cesium and rubidium, showing in each case that these new elements produced line spectra that were unique.”
Modern astrophysics was born with the development of spectroscopy and photography in combination with telescopes. For the first time, scientists could investigate what the universe was made of. The website of the American Institute of Physics (AIP) elaborates:
“In 1859, Bunsen reported to a colleague that Kirchhoff had made ‘a totally unexpected discovery.’ He had identified the cause of the dark lines seen in the solar spectra by Fraunhofer and others. When certain chemicals were heated in Bunsen’s burner, characteristic bright lines appeared. In some cases these were at exactly the same points in the spectrum as Fraunhofer’s dark lines. The bright lines were light coming from a hot gas, whereas the dark lines showed absorption of light in the cooler gas above the Sun’s surface. The two scientists found that every chemical element produces a unique spectrum. This provides a sort of ‘fingerprint’ which can confirm the presence of that chemical. Kirchhoff and Bunsen recognized that this could be a powerful tool for ‘the determination of the chemical composition of the Sun and the fixed stars.’ Throughout the 1860s, Kirchoff managed to identify some 16 different chemical elements among the hundreds of lines he recorded in the sun’s spectrum. From those data, Kirchoff speculated on the sun’s chemical composition as well as its structure. Early astronomical spectroscopy concentrated on the sun because of its brightness and its obvious importance to life on earth.”
The English astronomer Sir Joseph Norman Lockyer (1836—1920) and the French astronomer Pierre Janssen (1824—1907) are credited with discovering helium in 1868 through studies of the solar spectrum. Helium (from Greek helios for the Sun) is thus the only element (so far) discovered in space before being discovered on Earth.
The Englishman Sir William Huggins (1824-1910) was one of the most important early pioneers in astronomical spectroscopy. After 1875, some of his observations were made jointly with his astronomer wife Margaret Lindsay Huggins (1848-1915). After hearing about the discoveries made by Bunsen and Kirchhoff in 1859, Huggins took chemicals into the observatory to compare laboratory spectra with those of stars. Through spectroscopic methods he showed that stars are composed of the same elements as the Sun and the Earth. He explored the spectra of stars and nebulae, identified hydrocarbons in the spectra of several comets and was the first to attempt to measure the radial velocity of a star.
Photography provided a way of recording and preserving images of the spectra of stars. The Italian Jesuit priest and astrophysicist Pietro Secchi (1818-1878) in 1863 began collecting the spectra of stars, accumulating some 4,000 stellar spectrograms. He is considered the discoverer of the principle of stellar classification, although his system was later expanded into the Harvard classification system, which is based on a simple temperature sequence.
The Danish astronomer Ejnar Hertzsprung (1873-1967) and the American astronomer Henry Norris Russell (1877-1957) in the period 1911-1913 developed the Hertzsprung-Russell diagram, which relates the color of stars to their brightness. Herztsprung from Denmark studied photochemistry and applied his photographic skills to astronomical observations. He discovered the relationship between the brightness of a star and its color, but published these findings in a photographic journal which went largely unnoticed by astronomers. He was nevertheless offered a post by the great German Jewish physicist Karl Schwarzschild (1873-1916), who made valuable contributions to the understanding of relativity and black holes but sadly died on the Russian front during World War I. Hertzsprung moved with Schwarzschild to the Leiden Observatory in the Netherlands. Henry Norris Russell from New York made essentially the same discovery as Herztsprung, but published it in 1913 in a journal read by astronomers and presented the findings in a graph, which made them easier to understand. The temperature of a star is closely related to its color. The diagram helped give astrophysicists their first insight into the internal workings of the stars and their lifespan.
Many others made important contributions during the earliest phase of scientific spectroscopy. The German astronomer Hermann Karl Vogel (1841-1907) pioneered the use of the spectroscope in astronomy. Along with the American astronomer Edward Pickering (1846-1919), Vogel discovered spectroscopic binaries — double-star systems that are too close to be detected through direct observation with a telescope but which through the analysis of their light have been found to be two stars revolving around one another. The American astronomer Walter Sydney Adams (1876-1956) conducted spectroscopic studies of sunspots as well as the velocities and distances of thousands of stars.
This does of course not mean that spectroscopy replaced traditional, observational astronomy. The Englishman William Lassell (1799-1880) made good money from brewing beer and used some of it to indulge his interest in astronomy. In 1846 he discovered Triton, the largest moon of Neptune, shortly after that planet had itself been mathematically predicted by the French mathematician Urbain Le Verrier (1811-1877) and spotted by the German astronomer Johann Gottfried Galle (1812-1910) based on Le Verrier’s calculations. Lassel later discovered a satellite of Saturn, Hyperion, although this was done independently by American astronomers George Bond (1825-1865) and William Bond (1789-1859) as well. The American astronomer Asaph Hall (1829-1907) discovered the tiny moons of Mars (Deimos and Phobos) in 1877, and Richard Carrington (1826-1875), an English amateur astronomer, discovered by observing the motions of sunspots that the Sun rotates faster at the equator than near the poles.
Based on alleged minor disturbances in Neptune’s orbit, astronomers were looking for yet another unknown planet. The American astronomer Clyde Tombaugh (1906-1997) discovered Pluto in 1930 with photographic plates, which were used in serious astronomy and particle physics long after they had gone out of popular use, but by the twenty-first century even astronomers have switched to high-resolution digital cameras. The exploration of the outer reaches of our Solar System and beyond with better telescopes and cameras has challenged our previous knowledge of this region and the vocabulary used to describe it.
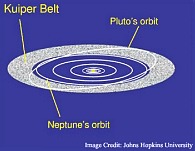
The dynamic but sparsely populated scattered disc is believed to be the source of many of the comets that sometimes visit our part of the Solar System. The entire trans-Neptune region is believed to be inhabited by primitive left-overs from the nebula of dust and gas that formed the Sun and the planets more than four and a half billion years ago. All the various objects that orbit the Sun at a greater average distance than the planet Neptune are collectively known as trans-Neptunian objects, or TNOs.
The scattered disc object Eris, which is believed to be slightly more massive than Pluto, was spotted in 2005 by a team led by Michael E. Brown (born 1965), professor of planetary astronomy at the California Institute of Technology. Anticipating the possibility that there could be more objects of similar size out there, the International Astronomical Union (IAU) in 2006 after heated discussions defined Eris as a “dwarf planet” along with Pluto, which consequently lost its position as the ninth planet of our Solar System. Brown’s team has discovered many distant bodies orbiting the Sun, among them the object Sedna in 2003.
According to the website of the Planetary Society, the existence of a belt of objects orbiting the outer reaches of our Solar System has been theorized since Pluto’s discovery in 1930:
“These objects would be primitive bodies, leftovers from the formation of the solar system, in a region too cold and sedate for planetary formation to proceed. For a long time Pluto and Charon were the only bodies known to inhabit this region of the solar system. But beginning in 1992 with the discovery of 1992 QB1, the observed population of this belt has grown almost to a thousand objects. The Kuiper belt spans a region of the solar system outside the orbit of Neptune, from about 30 to 50 Astronomical Units (AU). This region is close enough to Neptune that all of the Kuiper belt objects are considered to be under Neptune’s gravitational influence. Almost no objects have been observed beyond 50 AU, though astronomers should be able to detect them if they exist. The 50-AU boundary is referred to as the ‘Kuiper cliff.’ Whether the Kuiper cliff represents the outer boundary of the original planetary nebula, or whether it is merely the inner edge of a large ‘Kuiper gap’ extending at least to 70 or 80 AU, is not known. (The most distant known trans-Neptunian object, Sedna, has a perihelion of 76 AU, outside Neptune’s gravitational influence.)”
An Astronomical Unit (AU) roughly equals the distance between the Sun and the Earth, which is slightly less than 150 million kilometers. Neptune orbits the Sun at a distance of about 30 AU. One light year, i.e. the distance that light travels in vacuum in a year, is between nine and ten trillion (million times a million) kilometers, or more than 63 thousand AU. Our own Milky Way Galaxy is at least 100 thousand light years in diameter and our large galactic neighbor the Andromeda Galaxy is more than 2 million light years away. The Sun’s radius is approximately 109 times that of the Earth’s radius, it has 333 thousand times more mass than the Earth and contains well over 99% of the total known mass of our Solar System.
I could add that almost all of the numbers I quote here regarding the size of the universe are the result of research by Western astronomers. Although some of the ancient Greeks such as Eratosthenes and Hipparchus could work out realistic estimates for the size of the Earth and its distance to the Moon, which was in itself a major achievement and as far as I know unique among the ancient cultures, the true scale of our Solar System was worked out during the European astronomical revolution between the seventeenth and the nineteenth centuries.
The Dutch astronomer Jan Oort (1900-1992) theorized that the Sun is surrounded by a sphere of cometary nuclei. This so-called Oort cloud is believed to be exceedingly distant from the Sun, at 50 or perhaps 100 thousand AU, or more than a light year away. The latter figure is about a quarter of the distance between our Sun and the closest neighboring star and near the limit of the Sun’s gravitational pull. These objects can be affected by the gravity of other stars and be lost to interstellar space or pulled into the inner regions of our Solar System. The Estonian astronomer Ernst Öpik (1893-1985) suggested the existence of such a region already in 1932 whereas Jan Oort did so independently in 1950. For this reason, the Oort cloud is sometimes referred to as the Öpik-Oort cloud. It is believed that long-period comets, with orbits lasting thousands of years, originate here, but no Öpik-Oort cloud object has so far been directly observed. The body Sedna is too far away from Neptune to be influenced by its gravity and may have been affected by some unknown and very distant planetary-sized object, but as of 2009 this still remains speculations.
The study of electromagnetism was founded when the scholar Hans Christian Ørsted (1777-1851), sometimes called Oersted in scientific literature, from Denmark discovered in 1820 that there is a magnetic effect associated with an electric current. This announcement led to intensive research among scientists all over Europe, and the Frenchman André-Marie Ampère developed a mathematical form to represent the magnetic forces. The French physicist François Arago (1786—1853) found that when a magnetic compass needle was suspended by a thread over a copper disc and the disc was rotated, the needle was deflected. Arago was also influential in the development of the understanding of light and suggested in 1845 that Urbain Le Verrier investigate anomalies in the motion of Uranus, which led to the discovery of the planet Neptune in 1846. More groundbreaking advances in electromagnetism were made by the English physicist Michael Faraday (1791—1867). John Gribbin explains:
“The key experiment took place on 29 August 1831. To his surprise, Faraday noticed that the galvanometer needle flickered just as the first coil was connected to the battery, then fell back to zero. When the battery was being disconnected, it flickered again. When a steady electric current was flowing, producing a steady magnetic influence in the ring, there was no induced electric current. But during the brief moment when the electric current was changing (either up or down) and the magnetic influence was also changing (either increasing or decreasing) there was an induced current. In further experiments, Faraday soon found that moving a bar magnet in and out of a coil of wire was sufficient to make a current flow in the wire. He had discovered that just as moving electricity (a current flowing in a wire) induces magnetism in its vicinity, so a moving magnet induces an electric influence in its vicinity, a neatly symmetrical picture which explains Arago’s experiment, and also why nobody had ever been able to induce an electric current using static magnets. Along the way, having already in effect invented the electric motor, Faraday had now invented the electric generator, or dynamo.”
Faraday continued carrying out groundbreaking research in electricity and electrochemistry, popularizing terms such as “electrolyte,” “electrode,” “anode” and “cathode.” The development of various uses of electricity was rapid during the following generations. Late in the nineteenth century, electric trains were running in Germany, Britain and the USA. The Serb, and later American, inventor and electrical engineer Nikola Tesla (1856—1943) made many valuable contributions in the field of electricity, magnetism and radio, but the person who did the most to follow up Faraday’s investigations was the physicist James Clerk Maxwell from Edinburgh, Scotland. Faraday inspired Maxwell to study electromagnetism. He eventually concluded that light was a form of electromagnetic radiation. Before I explain that, however, I have to talk about measurements of the speed of light.
I have consulted many sources both online and in books, and as far as I can gather, no known scholar in any culture before seventeenth century Europe had ever made valid scientific measurements of the speed of either sound or light. In the eleventh century, the Persian scholar al-Biruni is said to have believed that the speed of light is much greater than the speed of sound, but this can easily be observed during a thunderstorm, when the flash is always seen before the bang. Alhazen is claimed to have stated that the speed of light is finite, but as long as no accurate measurements were made, this insight remained speculative.
Some European scholars during the Scientific Revolution still believed that the speed of light was infinite. As a matter of fact, light from the Sun takes more than eight minutes to reach the Earth. The first scientifically valid measurement of the speed of light which yielded a result that was at least in the right range was made by the astronomer Ole Christensen Rømer (1644-1710) from Denmark. Incidentally, he also developed one of the first scientific temperature scales. Daniel Gabriel Fahrenheit (1686—1736), a German physicist and maker of scientific instruments who spent most of his life in the Netherlands, visited Rømer in 1708 and improved on his temperature scale, the result being the Fahrenheit temperature scale still in use today in a few countries. In addition to this, Fahrenheit invented the mercury-in-glass thermometer, the first accurate, modern thermometer, in 1714.
The most widely used temperature scale is that created by Anders Celsius (1701-1744), a Swedish professor of astronomy at Uppsala University. In 1742 he proposed the temperature scale which now carried his name (Celsius himself called his scale centigrades), but he used 100 for the freezing point of water and 0 for the boiling point. The scale was reversed after his death by his countryman, the Swedish botanist Carl Linnaeus. Celsius also did research into the phenomenon known as aurora borealis and suggested that they were connected to changes in the magnetic field of the Earth.
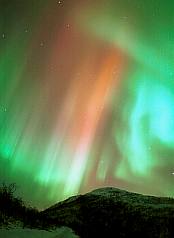
The first substantially correct theory of the origin of auroras, presented after the electromagnetic revolution, was created by the Norwegian physicist Kristian Birkeland (1867-1917). He undertook expeditions to study the aurora currents in the late 1890s and early 1900s and hypothesized that they were caused by the interaction of energetic particles from outside of the Earth’s the atmosphere with atoms of the upper atmosphere. Birkeland managed to reproduce in miniature the Solar System and could demonstrate the principles behind the auroras in his laboratory, yet his ideas were nevertheless rejected by most scientists of his time. In a drive to finance his expensive research he teamed up with the industrialist Samuel Eyde (1866-1940) and founded the company Norsk Hydro, inventing the first industrial scale method to extract nitrogen-based fertilizers from the air. However, by the 1920s this method was no longer able to compete with the German Haber-Bosch process.
The Swedish physicist Hannes Alfvén (1908-1995), one of the founders of plasma physics and magnetohydrodynamics, the study of plasmas in magnetic fields, supported Birkeland’s ideas, as did the pioneering Swedish scientist Svante Arrhenius (1859-1927), yet positive proof that Birkeland’s theory was correct was only obtained with the space age and observations made with satellites during the 1960s and 70s.
One large piece of the puzzle was the discovery of zones of highly energetic charged particles trapped in the Earth’s magnetic field. After the Soviet Union in October 1957 launched the first artificial satellite into orbit, Sputnik 1, the Americans launched their own Explorer 1 February in 1958. Its Geiger counter detected a powerful radiation belt surrounding the Earth. This was the first major scientific discovery of the space age. The belts were named the Van Allen radiation belts after the American space scientist James Van Allen (1914-2006). As we know today, there are actually two different radiation belts surrounding the Earth, and a third, weaker one which comes and goes depending on the level of solar activity.
The enormous conceptual leap that the speed of light, although very large, was not infinite, stemmed from the work of Ole Rømer. According to John Gribbin, “Rømer’s greatest piece of work was achieved as a result of his observations of the moons of Jupiter, carried out in conjunction with Giovanni Cassini (who lived from 1625 to 1712 and is best remembered for discovering a gap in the rings of Saturn, still known as the Cassini division)….Rømer predicted, on the basis of a pattern he had discovered in the way the eclipse times varied, that an eclipse of Jupiter’s innermost Galilean moon, Io, due on 9 November 1679, would occur ten minutes later than expected according to all earlier calculations, and he was sensationally proven right. Using the best estimate available of the diameter of the Earth’s orbit, Rømer calculated from this time delay that the speed of light must be (in modern units) 225,000 kilometres per second. Using the same calculation but plugging in the best modern estimate of the size of the Earth’s orbit, Rømer’s own observations give the speed of light as 298,000 kilometres per second. This is stunningly close to the modern value for the speed of light, 299,792 kilometres per second, given that it was the first measurement, ever, of this speed.”
Giovanni Cassini was a great Italian-born astronomer who spent much of his time as director of the Paris Observatory and thoroughly adopted his new home country of France. During the nineteenth century, these methods for measuring the speed of light were supplemented by increasingly precise non-astronomical measurements. Gribbin again:
“At the end of the 1840s, the French physicist Armand Fizeau (1819-1896)…had made the first really accurate ground-based measurement of the speed of light. He sent a beam of light through a gap (like the gaps in the battlements of a castle) in a rotating toothed wheel, along a path 8 kilometres long between the hilltop of Suresnes and Montmartre, off a mirror and back through another gap in the toothed wheel…. Fizeau was able to measure how long it took for light to make the journey, getting an estimate of its speed within 5 per cent of the modern determination….Léon Foucault (1819-1868), who had worked with Fizeau on scientific photography in the 1840s (they obtained the first detailed photographs of the surface of the Sun together), was also interested in measuring the speed of light and developed an experiment devised by Arago (and based on an idea by Wheatstone)….in 1850 Foucault first used this method to show (slightly before Fizeau did) that light travels more slowly in water than in air….By 1862 he had refined the experiment so much that he came up with a speed of 298,005 km/s, within 1 per cent of the modern value.”
That light travels more slowly through water than through air was a key prediction of all wave models of light, and more or less put to rest Newton’s theory of light as a stream of particles, at least for a while. The new, accurate measurements of the speed of light were invaluable for Maxwell’s theory of electromagnetism and light. In 1862 he calculated that the speed of light and the speed of propagation of an electromagnetic field are the same. His book Electricity and Magnetism appeared in 1873 and included the four partial differential equations known as Maxwell’s Equations. Soon after, the German physicist Heinrich Hertz (1857—1894) expanded and experimentally verified Maxwell’s electromagnetic theory of light. Hertz demonstrated the reality of radio waves in 1887. James E. McClellan and Harold Dorn:
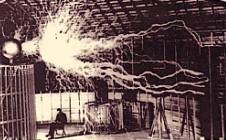
Marconi’s wireless telegraph was soon carried on a number of ships. The RMS Titanic, after it hit the iceberg and sank on 14 April 1912, had Marconi wireless radio operators calling for help from other ships. Sadly, in their case it was too late to avoid the tragedy which occurred.
Commercial radio broadcasts began in the 1920s. Radio astronomy was fully developed from the 1950s, but its successful practice goes as far back as 1932. Karl G. Jansky (1905—1950), an American radio engineer at the Bell Telephone Laboratories, was studying interference on the newly inaugurated trans-Atlantic radio-telephone service when he discovered that much of the interference came from extraterrestrial sources. After studying the phenomenon he concluded that much of the radiation was coming from the Milky Way. He published his findings in 1933, in the middle of the Great Depression. Grote Reber (1911—2002), an American amateur astronomer, built his own radio telescope in his back yard in 1937 and conducted the first sky survey in the radio frequencies. Further advances were made after the Second World War with pioneers such as John D. Kraus (1910—2004), an American physicist.
The German physicist Wilhelm Conrad Röntgen (1845—1923) discovered X-rays, electromagnetic radiation of extremely short wavelength and high frequency, in November 1895, which earned him the first Nobel Prize in Physics in 1901 and triggered a wave of interest which facilitated the discovery of radioactivity. X-rays are invisible to the human eye but affects photographic plates. The French physicist Paul Villard (1860-1934) discovered gamma rays in 1900 while studying uranium and radium. They have the highest frequency and energy and the shortest wavelength in the electromagnetic spectrum and can cause serious damage to living organisms. Gamma rays were first detected from astronomical sources in the 1960s. As with X-rays, gamma ray observations must preferably be made above the absorbing atmosphere of the Earth, which makes them well-suited for space telescopes.
As late as they year 1800, “light” still meant light that is visible to the human eye, nothing more. During the nineteenth century, our understanding of light was totally transformed into an electromagnetic spectrum stretching from radio waves via infrared radiation, visible light and ultraviolet radiation to X-rays and gamma rays. If we add the quantum revolution in the first three decades of the twentieth century, which I will describe soon, we can see that Europeans changed our understanding of light more in the space of just five generations than all civilizations had done combined in the previous five thousand years of recorded human history. This had major consequences as it was realized that visible light is only a small portion of the electromagnetic spectrum. By studying radiation in other wavelengths, astronomers have later uncovered new phenomena such as pulsars and quasars.
1 comments:
What about writing an essay "Spiritual optics"?
How to view a good guy, how to view a bad guy?
How to view a/the God?
Since your lenses are made of wavering psycho-materia, you are supposed to keep maximum quietude. No limits to that.
The Christian Greeks referred to "hesychia". That was their basis to build a monastery/observatory.
Also folk-tales provide many models how to view the good and evil. Surprisingly the paradigmata may change within the micro-epic.
The little folk tale draws a large picture of the evil getting subdued. The final view of the good is much more rewarding than (ever) expected.
The folk tale can be brutal, not its final effect.
Post a Comment